© 2023 MJH Life Sciences and Chromatography Online. All rights reserved.
© 2023 MJH Life Sciences™ and Chromatography Online. All rights reserved. Hplc Vials
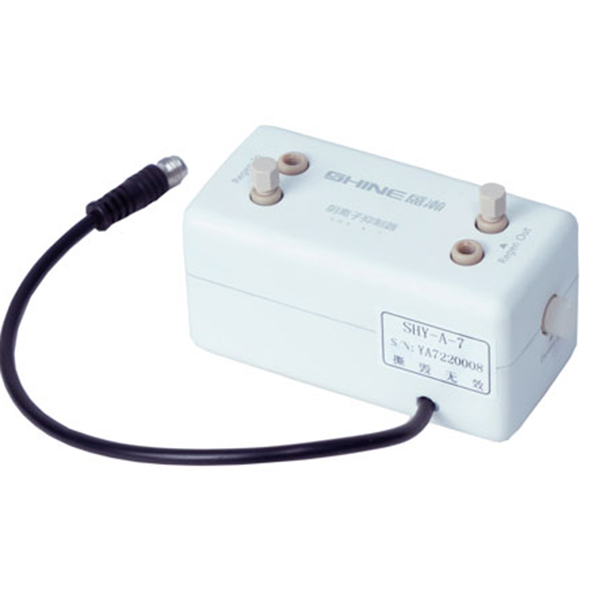
Metal-analyte interaction is a type of nonspecific adsorption (NSA) that occurs mainly between acidic analytes and active adsorptive sites present in the metal surfaces of the fluidic path of any chromatographic system. NSA represents a chromatographic challenge since it may lead to poor peak shape, low recovery, and poor performance from the high performance liquid chromatography (HPLC) system. As a result, many solutions that circumvent and mitigate this type of secondary interaction have been published or commercialized in the last few years. Such solutions encompass swapping stainless steel components for non-reactive polymers, “biocompatible” metals or alloys, the addition of additives into the mobile phase, pretreating the system with large amounts of sample or strong acids, and the use of coated stainless steel materials. This article explores these solutions in more detail, particularly the recent adaptation and benefits of coated metal surfaces in LC.
Stainless steel has been the preferred material to manufacture HPLC columns due to its ease of fabrication and mechanical resistance to pressure. Specifically, 316 stainless steel, which contains up to 3% molybdenum, has been implemented in instrument and column hardware due to its superior resistance to chemical corrodents, such as brine solutions and sulphuric acid. However, 316 stainless steel is still subject to corrosion and rust under certain conditions. The American Society for Testing and Materials International (ASTM International) official standard A967 has been the official protocol since 1996 describing the chemical treatment for stainless steel parts via nitric acid, citric acid, and electrochemical treatment. ASTM A967 also describes several tests to confirm the effectiveness of passivation, particularly concerning the removal of free iron. Furthermore, an “LCGC Troubleshooting” article, published soon after the ASTM A967 dossier, described the passivation and practical recommendations to keep a well-passivated high performance liquid chromatography (HPLC) instrument by using a 50% nitric acid solution as the last resource when stainless steel parts replacement is not a viable option (1).
Twenty-three years have passed since the issue of passivation was addressed in the LCGC article above, and, although only a handful of LC users would feel comfortable flushing 50% nitric acid solution through their system, new enabling and milder technologies have been reported in the literature (or even commercialized) to tackle stainless steel passivation from a chromatographic standpoint. However, now more than ever, stainless steel adsorption still represents a technical challenge that leads to poor recovery, poor peak shape, and even undetected analytes (2).
There are two proposed mechanisms for surface metal-analyte interaction. The first consists of trace metal impurities that are adsorbed by active sites within the fluidic flow path of the LC system, where they exhibit cation-exchange properties (3,4). The second is the electrostatic attraction of the negatively charged analytes to the electropositive metal surface (5). It is these strong interactions that lead to the chromatographic challenges and particularly for molecules prone to complexation and Coulombic interactions, such as phosphorylated compounds (for example, oligonucleotides), catecholamines (apomorphine), beta-hydroxy ketones (tetracyclines), and beta-hydroxy amines (salmeterol), among others (6).
This article will survey numerous passivating solutions, and their respective advantages and drawbacks, including leaching data from stainless-steel alternatives. It is worth mentioning that solutions labelled as “bioinert”, “biocompatible”, and “metal-free” are interchangeably (yet wrongly) used within the chromatography field. In general, the term “bioinert” refers to a surface that impedes adsorption, while the term “biocompatible” is used to define a corrosion-resistant material (7). Nevertheless, the LC user should be aware of such terminology when choosing some of the recent column technologies designed to mitigate nonspecific adsorption (8).
Table I summarizes the current solutions that have been developed by both academia and industry. As described in the previous section, acid treatment has been an established protocol for the passivation of stainless steel for many years. Nitric acid, citric acid, and phosphoric acid have mainly been the chemicals of choice, since they not only clean the surface by flushing out metal ions and other particulates but also improve the corrosion resistance by leaving a thin layer of chromium oxide on the surface of stainless steel (9). The goal is to achieve a higher ratio of chromium atoms to iron on the surface of the metal, rendering the surface passivated. Nevertheless, acid passivation is a temporary solution, as the corrosion process can and will start again once water, organic solvents, and salt additives are introduced into the system (10). Furthermore, proper care should be taken when performing acid passivation to make sure that all the strong acids are flushed out with water by performing a pH test, but also by making sure that all the parts of the HPLC systems are compatible with the strong acids, such as column compartments (LC phases) and detectors (flow cell or mass spectrometer). Improper system flushing and reconditioning with the mobile phase can lead to analyte suppression or damage to the instrument.
An alternate method to acid passivation is sample saturation of the surface’s active sites. This approach uses several injections of the analytes until a stable and reproducible signal is observed. The effectiveness of this temporary solution is dependent on the analyte’s affinity towards the oxide layer of stainless steel, the pH of the mobile phases, the isoelectric point of both the metal or alloy used and the molecule of interest, the overall surface area of the system’s wetted flow path, and concentration of the analyte. Hansen and associates determined the adsorption isotherms of 3,4-dihydroxybenzoic acid and compared it to that of the bovine serum albumin (BSA) and poly-L-Lysine. He concluded that BSA had the highest affinity towards stainless steel, an affinity of three orders of magnitude higher than the small molecule and just a bit higher than the oligopeptide (11). These determining factors ultimately impact the number of analyte injections required to obtain a reproducible signal, since these interactions are reversible and affected by other competing species within the sample or matrix.
Sometimes acid or sample passivation may not guarantee suitable chromatography, especially when dealing with trace metal ions constantly leaching from HPLC componentry or when the sample matrix is contaminated with metals. In such cases, metal chelators, either spiked into the sample or as part of the mobile phase, have been employed to sequester such metal ions flowing through the system. For example, citric acid and ethylenediaminetetraacetic acid (EDTA) are well-known metal chelators that are effective in improving the peak shape of many metal-sensitive analytes in both reversed-phase and normal-phase separations (12–14).
In recent years, medronic acid has been implemented for many liquid chromatography–mass spectrometry (LC–MS) analyses, including metal-sensitive applications, such as phosphorylated peptides and polar pesticide assays (15,16). Apart from solubility issues, the major drawback of such metal chelators is the propensity to interfere with signal sensitivity. In addition, metal-complexing acids can lead to signal suppression if used in high concentrations, since they could also be retained by the system, causing them to persist in future injections. However, when used correctly, medronic acid has shown minimal interference in metabolite-related applications and is commercialized as a mobile phase additive (17).
Polyetheretherketone (PEEK) polymer is a high-temperature thermoplastic that offers a unique combination of exceptional performance characteristics. It has been adapted in chromatography primarily as connecting tubing due to its chemical inertness, flexibility, easy-to-cut to any desired length, and ease of identification (colour coding). In addition, it can be finger tightened, economical, and is compatible with conventional HPLC pressures. On the other hand, PEEK’s most significant limitation comes from its inability to withstand ultrahigh-pressure liquid chromatography (UHPLC) pressures (18). From a manufacturing standpoint, internal diameters of PEEK tubing have shown vast variability in the past, which may result in retention and efficiency inconsistencies (19). Furthermore, PEEK may swell in the presence of chlorinated solvents, tetrahydrofuran (THF), and dimethyl sulfoxide.
PEEK tubing with stainless steel cladding offers a pressure advantage that the polymer alone cannot. It allows the polymer to withstand up to 18,000 psi pressures while covering the metal surface and preventing it from coming into contact with the flow path. However, to our knowledge, such a reinforcing technique only applies to tubing formats, leaving many other PEEK accessories, such as frits, unavailable. Figure 1 summarizes an internal study showing the theoretical plates per metre of several commercially available columns with similar particle technology and diameter. The stainless-steel clad columns have about 20–30% lower theoretical plates than the conventional columns. The lower performance could be attributed to PEEK’s internal diameter variability along the column length and the possible use of PEEK frits incompatible with highpressure column packing protocols. Although these drawbacks could be detrimental in specific cases, there is still merit in using PEEK hardware as a low-adsorptive and chemically inert solution for many HPLC applications (20).
Titanium and other biocompatible alloys have also been adopted as a solution for nonspecific adsorption. Many HPLC systems have been commercialized, with titanium pump heads and stators that guarantee excellent corrosion resistance against high salt concentrations and a wide pH range for biological applications. These instruments also come with many low adsorptive features, including ceramic needles, PEEK plumbing, stainless steel-cladding PEEK capillaries, and other metal alloys to maximize the benefits of the biocompatible metal. Several solutions are readily available in the column hardware field, including titanium filters, frits, and lining of the stainless-steel column tubing. Frits are particularly interesting since they are usually a high source of sample adsorption, due to their extensive surface area compared to the rest of the LC system (21,22).
Despite titanium’s high corrosion resistance, it was previously confirmed that it might be prone to pitting in anhydrous organic solvents, especially methanol and methanol-hydrogen chloride mixtures (23). Although titanium is considered a biocompatible metal, its metal surface can leach titanium ions into the flow path, increasing the chances of nonspecific adsorption. De Pra and associates found evidence that ciprofloxacin’s high peak asymmetry was attributed to titanium frits from the mobile phase reservoir. Titanium cations were leaching downstream, contaminating a polar-embedded stationary phase, chelating with ciprofloxacin, and causing severe peak tailing. The effect of titanium corrosion can be mitigated by adding a small amount of water to the organic solvent (4).
Another popular metal alloy commonly used in high-pressure systems is MP35N. This nickel-cobalt-based material (35% Ni, 35% Co, 20% Cr, and 10% Mo) also possesses much mechanical strength and anticorrosion characteristics needed for UHPLC; therefore, it is quite frequently used for tubing, autosampler needles, and valves (24). However, it has been found that this biocompatible alloy may also leach metal ions in the presence of several organic solvents, as indicated in a recent internal study where several samples of stainless steel, titanium, and MP35N alloy were assessed towards metal leaching (Figure 2).
Parts (a), (b), and (c) from Figure 2 correspond to the leached ions under methanol, water, and acetonitrile solvent. It is evident that the highest leaching effect resulted from methanol solvent, indicated by the amount of iron released from stainless steel, titanium ions from the titanium frit, and cobalt/nickel from MP35N. The second worst solvent for metal leaching was water, followed by acetonitrile. Titanium ions were not detected under water or acetonitrile conditions. It is worth noting that MP35N leaches the most metal ions of any alloy tested in this study when adjusted for surface area. The nonspecific adsorption consequence of appreciable amounts of cobalt and nickel within the flow path of chromatographic systems is still yet to be determined. However, nickel is widely used for immobilized metal affinity chromatography (IMAC) and the purification of proteins via nickel-histidine residue interactions (25,26).
Metal coatings have been essential in HPLC for quite some time, especially within injection valves. Diamond-like carbon (DLC), tungsten carbide, and other carbon composite materials have been deposited onto stators, increasing the lifetime of valves significantly when compared to uncoated ones. In addition, these coatings render the liquid-wetted surface low in absorptivity while maintaining compatibility with UHPLC pressures. However, some caveats persist in the coating technique. For example, DLC is, to some extent, a line-of-sight process, meaning substrate manipulation is necessary to ensure uniform deposition. However, due to the intricacies of the valve’s structure and drawbacks from the coating process, reduced adhesion could be achieved, which leads to delamination, oxidation, and overall poor lifetime performance (27).
Chemical vapour deposition (CVD) and its subtechnique, atomic layer deposition (ALD), are processes in which the substrate is exposed to one or more volatile precursor, which reacts with the heated substrate surface to produce the desired coating. Such non-line-of-sight techniques have been widely used in the semiconductor industry to create thin films while modifying optical, mechanical, electrical, and corrosion resistance attributes (28). In recent years, CVD-enabling technologies have found a niche within liquid chromatography columns and instrument components, therefore enhancing the analyses of many biologically relevant species such as oligonucleotides (5,29), drug metabolites (30), steroids (31), lipids (32), B-vitamins (33), peptides (34), monoclonal antibodies (35), and tricarboxylic acid cycle intermediates (36).
In an initial pilot study of our own, the peak height of three carboxylic acid-containing species (benzoic acid, 1,3,5-benzenetricarboxylic acid, and mellitic acid) was recorded. A standard HPLC system with an ultraviolet (UV) flow cell was used for this analysis, without prior system passivation or particular metal component optimization. The HPLC instrument and column underwent two different passivation methods as the injection sequence occurred. Initially, the analyte mixture was injected three times to establish a peak height baseline. Then, before the fourth injection, the instrument and column were passivated via three full-loop injections of medronic acid solution. After baseline equilibration, 10 more injections of the carboxylic acids were carried out while monitoring the peak heights. Lastly, before injection 14, the column was removed, CVD-coated ones replaced both inlet/outlet frits, and the analysis resumed once again for a total of 100 injections overall. Results are summarized in Figure 3.
At first glance, mellitic acid’s signal response change is drastic compared to the other two analytes, confirming the highest degree of nonspecific adsorption from mellitic acid towards the system’s metal surface. In addition, the abrupt increase of the analyte’s peak height after medronic acid injections demonstrates its passivation effect; however, the deterioration of medronic acid’s effect is observed after only 10 injections, bringing the intensity back to levels similar to prior passivation.
It is worth mentioning that the best passivation protocol for medronic acid is instead as a mobile phase additive in the low micromolar range to guarantee consistent performance with minimal to no signal suppression (12). However, highly concentrated injections were carried out for this internal study to observe the depletion of the passivation effect as the sequence proceeded. Once the stainless-steel column frits were exchanged for coated ones, the signal strength for all the analytes increased to levels even higher than the ones resulting from medronic acid. Furthermore, the peak heights remained relatively unchanged under the coated frits even after one hundred injections. These results highlight the metal-coated technology’s robustness and more prolonged effect and the significant and deleterious impact of column frits towards nonspecific adsorption compared to the rest of the instrument and column hardware.
Metal coating technologies offer a more consistent and robust alternative for the passivation of HPLC systems and column hardware by leveraging technologies used mainly in the semiconductor industry (37). Even though so-called “biocompatible” instruments and columns have been commercialized for several years through the implementation of primarily titanium and PEEK polymer hardware, coating-enabling technologies offer the low adsorption properties of an actual “bioinert” hardware without the drawbacks of pressure limitations, metal ion leaching, and possible lower column performance. Ultimately, these attributes will lead to more robust and reproducible chromatography while shortening the users’ time to acquire valuable and accurate data.
(1) Collins, K. E.; Collins, C. H.; Bertran, C. A. Stainless Steel Surfaces in LC Systems, Part II—Passivation and Practical Recommendations. LCGC North Am. 2000, 18 (7), 688–692.
(2) Hsiao, J. J.; Staples, G. O.; Stoll, D. R. Troubleshooting LC Separations of Biomolecules, Part 1: Background, and the Meaning of Inertness. LCGC Eur. 2020, 33 (3), 122–126.
(3) Euerby, M. R.; Johnson, C. M.; Rushin, I. D.; Sakunthala Tennekoon, D. A. S. Investigations into the Epimerisation of Tipredane Ethylsulphoxide Diastereoisomers during Chromatographic Analysis on Reversed-Phase Silica II. The Involvement of Metals in Commercially Available C18 Silicas. J. Chromatogr. A 1995, 705 (2), 229–245. DOI: 10.1016/0021-9673(95)00273-P
(4) De Pra, M.; Greco, G.; Krajewski, M. P.; et al. Effects of Titanium Contamination Caused by Iron-Free High-Performance Liquid Chromatography Systems on Peak Shape and Retention of Drugs with Chelating Properties. J. Chromatogr. A 2020, 1611, 460619. DOI: 10.1016/j.chroma.2019.460619
(5) DeLano, M.; Walter, T. H.; Lauber, M. A.; et al. Using Hybrid Organic–Inorganic Surface Technology to Mitigate Analyte Interactions with Metal Surfaces in UHPLC. Anal. Chem. 2021, 93 (14), 5773–5781. DOI: 10.1021/acs.analchem.0c05203
(6) Rainville, P.; Smith, K. Methods to Increase Sensitivity of LC/MS Analysis. US 11530966 B2, 2021.
(7) Fekete, S. Defining Material Used in Biopharmaceutical Analysis. LCGC Eur. 2021, 34 (6), 245–248. DOI: 10.56530/lcgc.eu.bq1982p9
(8) Bell, D. S. New Liquid Chromatography Columns and Accessories for 2022. LCGC Eur. 2022, 35 (5), 181–186. DOI: 10.56530/lcgc.na.sx2967r4
(9) Olefjord, I. The Passive State of Stainless Steels. Mater. Sci. Eng. 1980, 42, 161–171. DOI: 10.1016/0025-5416(80)90025-7
(10) Mowery, R. A., Jr. The Corrosion of 316 Stainless Steel in Process Liquid Chromatography with Acetonitrile or Methanol Carriers. J. Chromatogr. Sci. 1985, 23, 22–29. DOI: 10.1093/chromsci/23.1.22
(11) Hansen, D. C. Biological Interactions at Metal Surfaces. JOM 2011, 63, 22–27. DOI: 10.1007/s11837-011-0088-0
(12) McCalley, D. V. Influence of Metals in the Column or Instrument on Performance in Hydrophilic Interaction Liquid Chromatography. J. Chromatogr. A 2022, 1663, 462751. DOI: 10.1016/j.chroma.2021.46275
(13) Winter, D.; Seidler, J.; Ziv, Y.; Shiloh, Y.; Lehmann, W. D. Citrate Boosts the Performance of Phosphopeptide Analysis by UPLC-ESI-MS/MS. J. Proteome Res. 2008, 8, 418–424. DOI: 10.1021/pr800304n
(14) Than Myint, K.; Uehara, T.; Aoshima, K.; Oda, Y. Polar Anionic Metabolome Analysis by Nano-LC/MS with a Metal Chelating Agent. Anal. Chem. 2009, 81 (18), 7766–7772. DOI: 10.1021/ac901269h
(15) Birdsall, R. E.; Kellett, J.; Yu, Y. Q.; Chen, W. Application of Mobile Phase Additives to Reduce Metal-Ion Mediated Adsorption of Non-phosphorylated Peptides in RPLC/MSBased Assays. J. Chromatogr. B 2019, 1126–1127, 121773. DOI: 10.1016/j.jchromb.2019.121773
(16) Hsiao, J. J.; Potter, O. G.; Chu, T. W.; Yin, H. Improved LC/MS Methods for the Analysis of Metal-Sensitive Analytes Using Medronic Acid as a Mobile Phase Additive. Anal. Chem. 2018, 90 (15), 9457–9464. DOI: 10.1021/acs.analchem.8b02100
(17) Hsiao, J. J.; Yin, H.; Potter, O. G. Methods of Liquid Chromatography for Anionic Compounds. US 10620171 B2, 2017.
(18) Rivera, B.; Anspach, J. A.; Rao, S. Bioinert Versus Biocompatible: The Benefits of Different Column Materials in Liquid Chromatography Separations. LCGC Supplements 2018, 36 (6), 24–29.
(19) Hambleton, P.; Lough, W. J.; Maltas, J.; Mills, M. J. Unusual Analyte Adsorption Effects on Inert LC Components. J. Liq. Chromatogr. 1995, 18, 3205–3217. DOI: 10.1080/10826079508010445
(20) Murisier, A.; Fekete, S.; Guillarme, D.; D’Atri, V. The Importance of Being Metal-Free: The Critical Choice of Column Hardware for Size Exclusion Chromatography Coupled to High Resolution Mass Spectrometry. Anal. Chim. Acta 2021, 1183, 338987. DOI: 10.1016/j.aca.2021.338987
(21) Gritti, F.; Gilar, M. Impact of Frit Dispersion on Gradient Performance in High-Throughput Liquid Chromatography. J. Chromatogr. A 2019, 1591, 110–119. DOI: 10.1016/j.chroma.2019.01.021
(22) Sadek, P. C.; Carr, P. W.; Bowers, L. D.; Haddad, L. C. A Radiochemical Study of Irreversible Protein Loss on High-Performance Liquid Chromatography Column Frits. Anal. Biochem. 1985, 144, 128–131. DOI: 10.1016/0003-2697(85)90093-4
(23) Trasatti, S. P.; Sivieri, E. Corrosion Behaviour of Titanium in Non-Aqueous Solvents. Mater. Chem. Phys. 2005, 92, 475–479. DOI: 10.1016/j.matchemphys.2005.01.053
(24) Fehrenbach, T.; Wiese, S. Materials in HPLC and UHPLC—What to Use for Which Purpose. In The HPLC Expert II, 1st ed.; Kromidas, S., Ed.; Wiley-VCH, 2017; pp 223–267. DOI: 10.1002/9783527694945.ch7
(25) Alsaeedi, M.; Alghamdi, H.; Hayes, P.; et al. Evaluation of Hydrophilic Interaction Chromatography Versus Reversed-Phase Chromatography for Fast Aqueous Species Distribution Analysis of Nickel(II)-Histidine Complex Species. J. Chromatogr. A 2023, 1693, 463857. DOI: 10.1016/j.chroma.2023.463857
(26) Franken, K. L. M. C.; Hiemstra, H. S.; van Meigjaarden, K. E.; et al. Purification of His-Tagged Proteins by Immobilized Chelate Affinity Chromatography: The Benefits from the Use of Organic Solvent. Protein Expression Purif. 2000, 18, 95–99. DOI: 10.1006/prep.1999.1162
(27) Delfani-Abbariki, S.; Abdollah-zadeh, A.; Hadavi, S. M. M.; Abedi, M.; Derakhshandeh, S. M. R. Enhancing the Adhesion of Diamond-like Carbon Films to Steel Substrates Using Silicon-Containing Interlayers. Surf. Coat. Technol. 2018, 350, 74–83. DOI: 10.1016/j.surfcoat.2018.06.055
(28) Behera, A.; Mallick, P.; Mohapatra, S. S. Chapter 13 - Nanocoatings for Anticorrosion: An Introduction. In Micro and Nano Technologies, Corrosion Protection at the Nanoscale; Elsevier, 2020; pp 227–243. DOI: 10.1016/B978-0-12-819359-4.00013-1
(29) Gilar, M.; DeLano, M.; Gritti, F. Mitigation of Analyte Loss on Metal Surfaces in Liquid Chromatography. J. Chromatogr. A 2021, 1650, 462247. DOI: 10.1016/j.chroma.2021.462247
(30) Plumb, R. S.; Gethings, L. A.; King, A.; et al. Hybrid Organic/Inorganic Hybrid Surface Technology for Increasing the Performance of LC/MS(MS)-Based Drug Metabolite Identification Studies: Application to Gefitinib and Metabolites in Mouse Plasma and Urine. J. Pharm. Biomed. Anal. 2021, 200, 114076. DOI: 10.1016/j.jpba.2021.114076
(31) Tanna, N.; Mullin, L. G.; Rainville, P. D.; Wilson, I. D.; Plumb, R. S. Improving LC/MS/MS-Based Bioanalytical Method Performance and Sensitivity via a Hybrid Surface Barrier to Mitigate Analyte–Metal Surface Interactions. J. Chromatogr. B 2021, 1179, 22825. DOI: 10.1016/j.jchromb.2021.122825
(32) Isaac, G.; Wilson, I. D.; Plumb, R. S. Application of Hybrid Surface Technology for Improving Sensitivity and Peak Shape of Phosphorylated Lipids such as Phosphatidic Acid and Phosphatidylserine. J. Chromatogr. A 2022, 1669, 462921. DOI: 10.1016/j.chroma.2022.462921
(33) Yang, J.; Wilson, I.; Rainville, P. Evaluation of Hybrid Surface Technology for the Analysis of the B-Group Vitamins by LC-ESI-MS/MS. J. Chromatogr. B 2022, 1204, 123336. DOI: 10.1016/j.jchromb.2022.123336
(34) Hughes, C. J.; Gethings, L. A.; Wilson, I. D.; Plumb, R. S. Access to the Phospho-proteome via the Mitigation of Peptide-Metal Interactions. J. Chromatogr. A 2022, 1673, 463024. DOI: 10.1016/j.chroma.2022.463024
(35) Fekete, S.; DeLano, M.; Harrison, A. B.; et al. Size Exclusion and Ion Exchange Chromatographic Hardware Modified with a Hydrophilic Hybrid Surface. Anal. Chem. 2022, 94 (7), 3360–3367. DOI: 10.1021/acs.analchem.1c05466
(36) Smith, K. M.; Wilson, I. D.; Rainville, P. D. Sensitive and Reproducible Mass SpectrometryCompatible RP-UHPLC Analysis of Tricarboxylic Acid Cycle and Related Metabolites in Biological Fluids: Application to Human Urine. Anal. Chem. 2020, 93 (2), 1009–1015. DOI: 10.1021/acs.analchem.0c03863
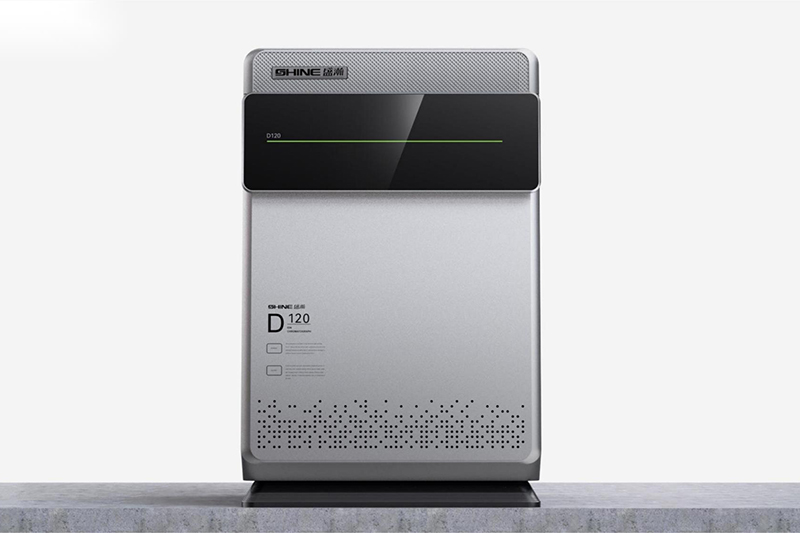
Anion Exchange Chromatography (37) Mittal, M.; Sardar, S.; Jana, A. Nanofabrication Techniques for Semiconductor Chemical Sensors. In Micro and Nano Technologies, Handbook of Nanomaterials for Sensing Applications, 1st ed.; Hussain, C. M., Kailasa, S. K., Eds.; Elsevier, 2021; pp 119–137. DOI: 10.1016/B978-0-12-820783-3.00023-3